Earth and Venus are about the same size and weight. Therefore planetary geologists assume they are probably very similar internally in terms of their core, mantle and crust. However, Earth has an extensive system of mid-ocean ridges that are long skinny mountain chains in the ocean basins that mark where hot magma (molten rock) erupted at the surface to create new ocean floor as the continents drifted or spread apart . It also has mountain chains, such as the Cordillera and the Andes along the west coast of the American continents, where the ocean floor dives down or subducts beneath the continents, and the Himalayas that formed where the Indian subcontinent collided with the rest of Asia. This is the typical planetary signature of what’s called “Plate Tectonics”, the process of continental drift The important thing to understand here is that plate tectonics is the dominant way the Earth as a planet cools its interior, and this is critical for understanding the planetary geology of the “Rocky Planets”.
“Rocky Planets” contain radioactive elements such as U (that breaks down to Pb) and K (that breaks down to Argon). Radioactive breakdown to Pb) and K (that breaks down to Argon). Radioactive breakdown releases energy that turns into heat inside planets. Therefore, if relatively large planets like Venus and Earth do not have an efficient cooling mechanism, they will accumulate that heat inside, and the planetary interior can get so hot that it can melt with potentially catastrophic consequences! However, Venus has no ocean basins, no continents, no spreading ridges, no subduction zones, no collisional mountain belts. If Venus is made of the same stuff as the Earth, it probably contains radioactive elements that produce heat , so why doesn’t it look geologically like the Earth?
80% of the Venusian surface is made of gently rolling plains of volcanic rock, dotted with large isolated volcanoes, and remarkably few impact craters! What does this tell us? The low number of impact craters indicates that the surface of Venus is younger than 3.8 billion years old, and the volcanic surface tells us that the original impact craters were swamped by a huge volume of lava that completely resurfaced the planet on a global scale, somewhat akin to resurfacing a road to cover over the potholes. By studying the number and size of the impact craters, planetary geologists estimate that this planetary resurfacing occurred sometime between 500 million and 1 billion years ago. Although that still sounds like a long time ago, it’s relatively young compared to the end of the Great Bombardment, 3.8 billion years ago.
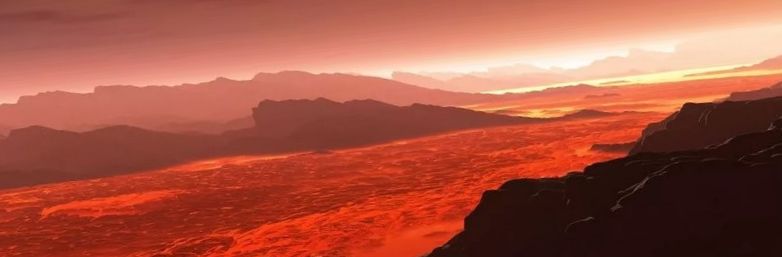
Plate tectonics allows planet Earth to cool. However, Earth also has plenty of water, but Venus doesn’t, and it’s water that allows plate tectonics to operate on Earth. In fact, Venus has no water inside either, but how do we know that? Some Venusian volcanoes are rather peculiar, compared with those on Earth. They have very flat tops and very steep sides, about a kilometre high. They look like pancakes and must have formed when very stiff and sticky lava flowed out from the central vents, otherwise the sides of the volcanoes wouldn’t be so steep. They also lack impact craters: hence, these volcanoes are relatively young. So, why is this Venusian lava so stiff?. It all comes back to water! Rocks are made of silicate minerals like quartz, feldspar, olivine and pyroxene. Silicate lavas are very sticky and stiff when they are dry, but flow like treacle when they are wet, so the pancake volcanoes on Venus were probably built by very dry lavas. Solid rock can also flow … if it is hot enough, and given enough time. However, if the rock is very dry, then it’s very strong and stiff and can hardly flow at all. In contrast to Venus, Earth has water at the surface that gets carried into its warm interior by the ocean floor material that slides down the subduction zones. This makes the Earth’s interior soft enough to flow, thereby allowing the plates to move and letting hot mantle material rise up under the mid-ocean ridges. The consequence is that the Earth’s interior is able to cool, albeit very slowly, but dry Venus, with its stiff interior cannot flow, so plates can’t move and heat cannot escape. The radioactive heat builds up on a time scale of 100’s of millions of years until the upper part of the planetary interior melts. Molten lava, even dry, is still softer than solid dry rock, so upward movement of lava can take place and quickly resurface the entire planet in a geologically short time. This rapid turnover of the upper part of the planetary interior allows heat to escape, things cool off and stabilise, and the planetary interior returns to its normal strong, stiff state that cannot flow fast enough to allow tectonic plates to form and move around. Meanwhile, radioactive heat continues to be produced until the next resurfacing event occurs 100’s of millions of years in the future.