What is Spectroscopy? Spectroscopy is the study of the cosmos through the composition of light. Natural processes leave a characteristic ‘fingerprint’ encoded in the light that we observe. Images tell us where to look for interesting new discoveries. Spectroscopy explains what we are seeing. The electromagnetic spectrum can be expressed in terms of energy, wavelength, or frequency. In nature there are various different processes which emit electromagnetic radiation. Anywhere charged particles are being accelerated, light is emitted. The frequency tells us about the energy of the source.
Table of Contents
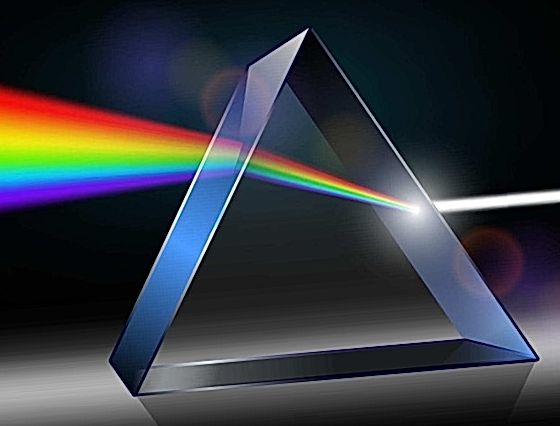
Thermal Radiation
Every solid object vibrates at the molecular level. These oscillations result in thermal radiation. The wavelength of the peak emission depends on the temperature of the object. This is why a ‘white-hot’ object is hotter than a ‘red-hot’ object. Room temperature objects have a peak emission at ~10 m in the infrared.
Types of Spectra
Free floating atoms and molecules in a gas will only vibrate at specific frequencies, giving rise to line spectra: effectively a finger print which is unique to each chemical species. Hot gasses emit while cold gasses absorb. Stars are classified by their spectra.
The OBAFGKM classification scheme breaks down roughly as a function of temperature. The line spectra, superimposed on the stellar thermal emission tell us about the ‘cool’ gaseous elements and molecules on the surface of the star.
The Hertzsprung-Russel Diagram
By plotting temperature against brightness, we can calculate a star’s size even though we can’t resolve it’s disc (stars of identical size fall on the dashed diagonal lines).
Most stars appear on the ‘Main Sequence’. What about dark dust and gas? Can we somehow measure the fingerprint of dark clouds? The way that dust ‘reddens’ starlight tells us about the size of the intervening particles. The ‘reddened’ star is the same spectral type, but is partially obscured by a cloud of cold dust and gas.
We know it’s the same type even though the thermal spectrum appears reddened (due to foreground dust). This is because the same stellar absorption lines are present in the spectrum indicating a similar surface temperature. The strongest DIBs were first noted in the 1920s. These bands in the visible region represent the longest unsolved problem in spectroscopy. Prior to the ’70s, astronomers used to think that molecules couldn’t survive the harsh UV environment of space. Since then, large radio telescopes have detected the fingerprint of complex molecules in dark clouds, some up to 13 atoms in size. New laboratory work suggests that the DIBs are associated with complex carbonaceous (organic) molecules and ions.
Complex organic molecules have been identified in space based on their fingerprint! It is likely that these molecules are formed in dense clouds, perhaps through surface-catalyzed reactions on dust grains, where they are protected from the UV flux until they reach sizes where photo-dissociation in diffuse interstellar regions becomes inefficient.
The fingerprint of Buckminsterfullerene is found amongst the DIBs. (Foing Erhenfreund 1997) Mixture of PAH cations are found in the Orion bar. These PAH molecules are commonly found in tar, coal, auto exhaust and BBQ grills.
NASA/Ames Astrochemistry Pre-biotic chemistry results in a cornucopia of organic molecules in the harsh, low-density environment of space, all recognized by their electromagnetic fingerprint.
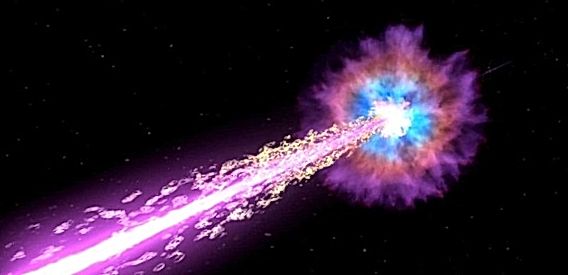
In the molecular clouds obscuring Sagittarius B2, ethanol has been measured, using large ground-based radio telescopes, at a density of ~1 molecule/m3. This may not seem like much, but when you consider just one typical cloud with a 5 parsec radius, there’s enough alcohol to supply every man, woman and child on earth with 1 billion pints of ice cold beer each, every second, for the extent of their natural lives! Unfortunately, there’s also some pretty nasty stuff out there like methanol and formaldehyde which would result in a rotten hangover. Astrochemists have determined that this is actually grain alcohol. The interstellar gas cannot produce the observed densities faster than they are destroyed by UV light. The alcohol can only be produced on the surface of dust grains at the required rates. There is also plenty of water in interstellar clouds. The Submillimeter Wave Astronomy Satellite has discovered that the ratio of alcohol to cold water in some regions of Sgr B2 ranges up to 2%, suggesting that any beer one might make, if one were to condense these clouds, would be sold in the US.
The Mystery of Gamma-Ray Bursts (GRBs)
Rivalling energy levels of the Big Bang, gamma ray bursts are the most luminous (and powerful) point sources in the observable universe. Hundreds of times brighter than your typical super nova, these bursts are detected about once or twice from random directions in the sky (on a daily basis). After the initial burst of gamma-rays come longer periods of diminishing energy in x-ray, ultra-violet, optical and radio wavelengths. Astronomers call this phenomena the optical afterglow or counterpart. Gamma-ray bursts have been recorded in bunches, followed by periods of inactivity.
Anatomy of a GRB
Not all gamma-rays are ‘seen’ or detected. Most optical jets are not aligned with the Earth. If astronomers would be able to record every event, then about thirty bursts a second would be detected.
Gamma-ray bursts come in two flavours. There are the short ones that last less than two seconds with an average duration time of 0.3 seconds. The long bursts are more than two seconds with an average duration time of roughly 30 seconds. Collapsar/Hypernova Model
Scientists discovered GRBs by accident in 1967 when military satellites detected residual gamma-rays from space. Initially, these ‘Vela’ satellites were used to detect gamma-rays from Soviet nuclear tests above or below the ground.
Then is 1973, scientists at the Los Alamos National Laboratory use this data to determine they originated from deep space. At first they assumed that GRBs came from our Milky Way or even just beyond the confines of the solar system. Even as late as the early 1990’s astronomers were still unsure where they came from.
Satellite observations from the now decommissioned Comption Gamma-Ray Observatory and the joint Italian/Dutch consortium which launched the BeppoSAX probe enabled astronomers to develop a working model of the type of star that produces a long GRB.
This type of star is called a “hypernova.” It is a rare type of a supercharged super-nova from an extremely massive star that undergoes core collapse. These gargantuan stars are at least 100 solar masses are situated at cosmological distances. A blast-wave of material is produced inside a collapsing star, also called a “collapsar.” A black hole forms inside the star before it detonates. The material plows through the star and heats the internal layers to millions of degrees. The ensuing heat and energy create the gama-rays.
The gamma-rays and the blast-wave of stellar material then erupt from the star. High energy gamma-rays will travel at light speed while the stellar material will move a little slower. Both types of radiation will travel along a specific axis. This material will form an expanding cone that will collide with dust and gas in the interstellar medium producing a less energetic afterglow in x-ray, ultra-violet, optical and radio wavelengths. Also note that the spin energy or angular momentum from the spinning black hole is converted into hard radiation that comes out as gamma-rays as well.
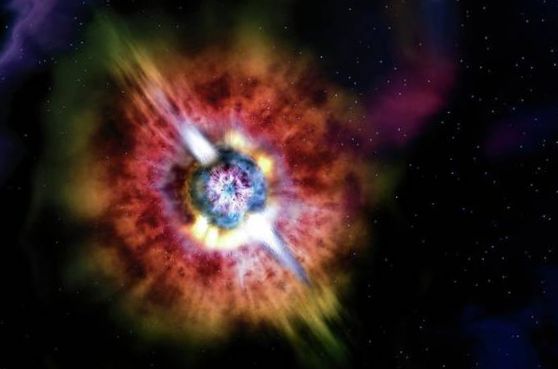
If our planet happens to cross one of those beams, instruments in space will detect a brief pulse of gamma-rays.
However, it is the short bursts, those lasting less than two seconds, that still remain a bit of a mystery. Up until recently, telescopes were simply too slow to respond to them. The optical counterparts are too fleeting. Without gaining any data from their afterglows, a GRB’s location, distance and physical structure cannot be had. Observing the optical counterpart is crucial as far as finding out the nature of these beasts.
Their relative proximity to Earth has astronomers favouring the neutron star/neutron star merger scenario. A less popular theory features the neutron star/black hole encounter.
These events happen in older, redder elliptical galaxies where these objects exist. Neutron star mergers take a long time to evolve and develop, therefore these collisions occur in ‘recent’ times. The bottom line… preliminary data favour the compact-object merger theory for short duration GRBs. Now in Earth orbit, the ‘Swift’ spaceprobe has provided much information about these elusive objects. It’s two-year mission is to study and observe GRBs in great detail. As of June 2006, ‘Swift’ has detected at least 120 GRBs with more exciting science to come. As soon as gamma-ray photons hit it’s detector, it responds almost instantly (about 30 seconds) by slewing the scope at the GRB source. The Burst-Alert Telescope (BAT) can pick-up as little as eleven gamma ray photons and send this data to activate the X-Ray Telescope (XRT) and the Ultra-violet Optical Telescope (UVOT). Unlike the Hubble telescope, ‘Swift’ can respond literally in seconds.
About 900 light years from here, there’s a rocky planet not much bigger than Earth. It goes around its star once every hundred days, a trifle fast, but not too different from a standard Earth-year. At least two and possibly three other planets circle the same star, forming a complete solar system.
The star is a pulsar, PSR 1257+12, the seething-hot core of a supernova that exploded millions of years ago. Its planets are bathed not in gentle, life-giving sunshine but instead a blistering torrent of X-rays and high-energy particles.
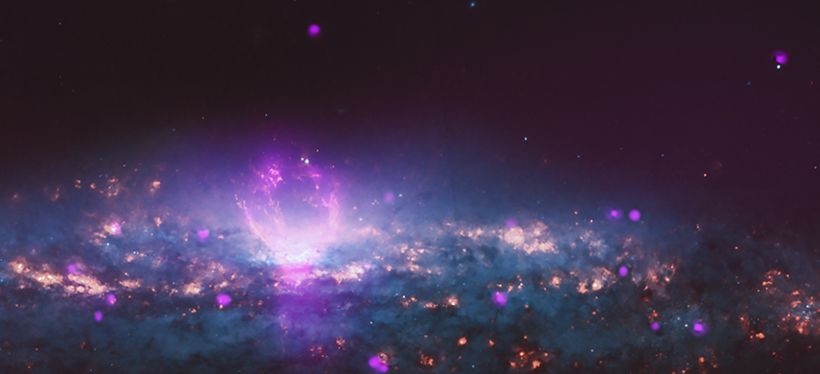
“It would be like trying to live next to Chernobyl,” says Charles Beichman, a scientist at JPL and director of the Michelson Science Center at Caltech. Our own sun emits small amounts of pulsar-like X-rays and high energy particles, but the amount of such radiation coming from a pulsar is “orders of magnitude more,” he says. Even for a planet orbiting as far out as the Earth, this radiation could blow away the planet’s atmosphere, and even vaporize sand right off the planet’s surface.
Astronomer Alex Wolszczan discovered planets around PSR 1257+12 in the 1990s using Puerto Rico’s giant Arecibo radio telescope. At first, no one believed worlds could form around pulsars it was too bizarre. Supernovas were supposed to destroy planets, not create them. Where did these worlds come from?
NASA’s Spitzer Space Telescope may have found the solution. Last year, a group of astronomers led by Deepto Chakrabarty of MIT pointed the infrared telescope toward pulsar 4U 0142+61. Data revealed a disk of gas and dust surrounding the central star, probably wreckage from the supernova. It was just the sort of disk that could coalesce to form planets!
As deadly as pulsar planets are, they might also be hauntingly beautiful. The vaporized matter rising from the planets’ surfaces could be ionized by the incoming radiation, creating colorful auroras across the sky. And though the pulsar would only appear as a tiny dot in the sky (the pulsar itself is only 20-40 km across), it would be enshrouded in a hazy glow of light emitted by radiation particles as they curve in the pulsar’s strong magnetic field.
Wasted beauty? Maybe. Beichman points out the positive: “It’s an awful place to try and form planets, but if you can do it there, you can do it anywhere.”